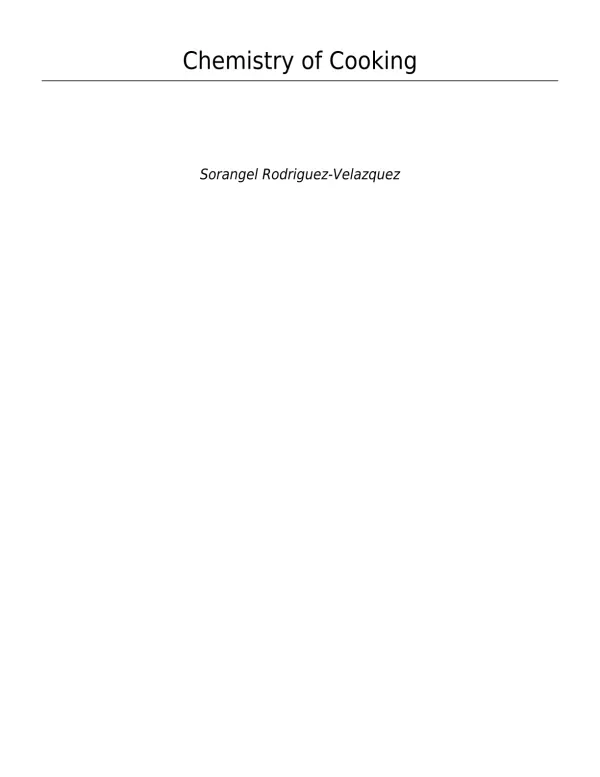
Cooking Chemistry: Ingredients & Techniques
Document information
Author | Sorangel Rodriguez-Velazquez |
Major | Chemistry |
Document type | Textbook |
Language | English |
Format | |
Size | 16.23 MB |
Summary
I.The Chemistry of Everyday Life and Cooking
This section explores the fundamental role of chemistry in everyday activities, focusing on the chemistry of cooking. From brewing coffee and baking bread to the ripening of bananas and the making of caramel, countless daily processes involve chemical substances and reactions. Understanding these principles is crucial for appreciating the science behind food preparation. Key concepts include chemical reactions, physical and chemical properties of ingredients, and the transformation of matter during cooking.
1. Chemistry s Ubiquitous Role in Daily Life
The introductory section emphasizes the pervasive presence of chemistry in everyday life, extending far beyond the confines of a formal academic setting. It highlights the chemical processes inherent in common activities such as making coffee, cooking eggs, and even the ripening of bananas. The preparation of food is presented as a prime example of applied chemistry, with each step, from selecting ingredients (flour, sugar, butter) to employing cooking technology, involving chemical substances and reactions. This section establishes the course's aim to unravel the fundamental chemical principles underlying the art of cooking, underscoring the practical and relevant nature of chemistry in our daily experiences. The text explicitly states, "Whether you are aware or not, chemistry is part of your everyday food. In this course, you will learn many of the essential principles underlying the chemistry of cooking." This sets the stage for a detailed exploration of culinary chemistry, promising to make the subject engaging and relatable by connecting abstract chemical concepts to familiar, everyday occurrences. The reader is subtly prompted to reflect on their daily routines and the surprisingly vast number of chemical processes involved, thereby fostering a sense of wonder and interest in the subject.
2. Chemical Processes in Food Preparation Specific Examples
This section provides concrete examples of chemical transformations occurring during food preparation. The text specifically mentions making coffee, cooking eggs, and toasting bread as processes that involve chemistry. It further expands this idea by discussing the chemical substances and processes involved in more complex preparations. The examples given range from the relatively simple (e.g., toasting bread) to more elaborate culinary actions such as waiting for bananas to ripen or melting sugar to make caramel. Each of these actions is presented as an illustration of chemical substances and processes, demonstrating that cooking is essentially a series of chemical reactions and transformations, making it a highly practical application of chemistry in our daily lives. The importance of understanding these underlying chemical principles is emphasized to provide a deeper appreciation of cooking techniques and the resulting food properties. This section aims to bridge the gap between abstract chemical principles and the concrete experience of preparing food, making the study of chemistry more tangible and engaging for the reader.
3. Chemistry Beyond the Kitchen A Widespread Influence
The section broadens its scope beyond culinary chemistry to emphasize the pervasiveness of chemical principles in various aspects of modern life. It uses the scenario of a typical morning routine – waking up, showering, commuting, and grabbing a coffee and breakfast sandwich – to illustrate that seemingly mundane activities are filled with chemical transformations. It then presents the question, "Why should we study chemistry?", directly engaging the reader to consider the significance of chemistry in their own lives. The illustrative morning routine serves as a powerful hook to highlight the integration of chemistry into our daily experiences, whether we are consciously aware of it or not. The text implies that chemistry is not merely a theoretical subject confined to textbooks, but a practical science with tangible real-world consequences that impact everything from our personal routines to technology. The transition from everyday actions to the central question of the importance of studying chemistry underscores the practical relevance of chemical principles and their impact on our daily lives.
II.The Law of Conservation of Matter
This section delves into the law of conservation of matter, demonstrating its relevance through examples such as beer brewing and lead-acid battery operation. The principle states that matter cannot be created or destroyed, only transformed. This concept is a cornerstone of chemistry, crucial for understanding chemical reactions and physical changes.
1. The Law of Conservation of Matter Core Principle and Examples
This section introduces the fundamental principle of the law of conservation of matter, stating that matter is neither created nor destroyed in chemical reactions; it merely undergoes transformation. The text emphasizes that the total mass remains constant throughout a chemical process. Two compelling real-world examples are presented to illustrate this concept: the brewing of beer and the operation of a lead-acid car battery. In the beer-brewing process, the initial ingredients—water, yeast, grains, malt, hops, and sugar—are converted into the final product, beer (water, alcohol, carbonation, and flavoring substances), without any loss of mass. The transformation of glucose into ethanol and carbon dioxide during bottling is highlighted as a clear demonstration of this principle. Similarly, a lead-acid battery's function is explained as a chemical transformation where the original substances (lead, lead oxide, and sulfuric acid) change into other substances (lead sulfate and water), again without any alteration in the total mass. These everyday examples serve to effectively ground the abstract concept of conservation of matter in tangible, relatable contexts, enhancing understanding and appreciation of this crucial chemical principle.
2. Expanding on Dalton s Atomic Theory
The section briefly touches upon John Dalton's atomic theory, acknowledging that while some aspects have been refined over time, many elements of the theory remain valid. This serves as a concise transition to subsequent discussions of atomic structure and behavior. The text mentions that more detailed information about Dalton's theory can be found in a later chapter focused on atoms and molecules. While this portion does not delve deeply into specific details of the theory or its modifications, the mention of Dalton's work provides a historical and conceptual link to the ongoing relevance of his contributions to the understanding of matter. It also effectively sets the stage for further exploration of atomic-level processes that underpin the macroscopic law of conservation of matter. This establishes a clear trajectory for the reader, foreshadowing future discussions that will offer a more detailed and nuanced understanding of the underlying mechanisms responsible for conservation of mass during chemical transformations.
3. Addressing the Law s Applicability in Real World Scenarios
The final part of this section presents a series of scenarios to test the reader's understanding of the law of conservation of matter, moving beyond the initially presented examples of beer brewing and lead-acid batteries. The questions ask whether the mass would increase, decrease, or remain constant during specific chemical reactions. These scenarios include baking bread, burning magnesium in air, and Antoine Lavoisier's classic experiment with tin and air in a sealed flask. The inclusion of these questions encourages active engagement with the material and reinforces the understanding of the law's implications in various practical contexts. By presenting these real-world scenarios, the section effectively bridges the gap between the theoretical understanding of the law and its practical applications, solidifying the reader's grasp of this fundamental concept in chemistry. The questions also implicitly address the occasional complexities of observing the law in everyday situations, suggesting that while the principle holds true, practical applications may involve subtle factors that require a deeper understanding of chemical processes.
III.Atoms Molecules and Chemical Bonding
This section introduces the building blocks of matter: atoms and molecules. It explains the concept of chemical bonds that hold atoms together in molecules, illustrating the incredibly small scale of these particles using comparisons like a spiderweb and golf balls. The section also differentiates between elements and compounds and introduces the concept of isomers, molecules with the same chemical formula but different structures.
1. Introducing Atoms and Molecules Size and Scale
This section begins by introducing the concepts of atoms and molecules, emphasizing their incredibly small size. To illustrate this point, the text uses the analogy of a spiderweb thread, which, while visible to the naked eye, is still vastly larger than a single atom. A comparison is made between the diameter of a spiderweb thread (approximately 0.00001 cm) and that of a carbon atom (approximately 0.000000015 cm), highlighting the immense difference in scale. The text further enhances the understanding of these microscopic dimensions by employing another analogy: if a carbon atom were the size of a dime, the cross-section of a single spiderweb thread would be larger than a football field. This illustrates the vast difference in scale between macroscopic objects and the atomic realm. By utilizing these relatable comparisons, the section successfully conveys the conceptual difficulty of grasping the minuscule dimensions of atoms and molecules, while also emphasizing the immense number of atoms present even in seemingly small objects. The section's use of analogies serves as a powerful teaching tool, making the abstract concept of atomic and molecular size more concrete and accessible to readers.
2. Molecules Structure and Composition
Building on the introduction of atoms and molecules, this section defines molecules as units composed of two or more atoms joined by strong forces called chemical bonds. It explains that atoms in a molecule move as a unit, using analogies like a six-pack of soda cans or a bunch of keys on a keyring. The text also distinguishes between molecules composed of identical atoms (like those in hydrogen, oxygen, and sulfur) and molecules consisting of different atoms (like water, with two hydrogen and one oxygen atom, or glucose, with six carbon, twelve hydrogen, and six oxygen atoms). The section further reinforces the concept of molecular scale by comparing the size of water molecules to golf balls if a glass of water were enlarged to the size of Earth. This provides a tangible way to grasp the minuscule size of molecules. The detailed description of molecular structure, the different ways atoms can combine, and the use of evocative comparisons help make this fundamental chemical concept more intuitive and accessible.
3. Elements Compounds and Chemical Changes
The section introduces the distinction between elements and compounds. It defines compounds as pure substances that can be broken down into simpler substances (elements or other compounds) through chemical changes. Several examples are given to illustrate this concept: mercury(II) oxide decomposing into mercury and oxygen by heating; sucrose breaking down into carbon and water; and silver(I) chloride decomposing into silver and chlorine upon exposure to light. The process of caramelization is mentioned as the initial stage of sucrose decomposition, linking chemistry to a familiar culinary process. The use of silver(I) chloride in photographic films and photochromic eyeglasses is cited as an example of a practical application of this chemical property. By providing clear examples and linking them to everyday objects and processes, the section effectively illustrates the concept of compounds and their decomposition, solidifying the understanding of chemical changes and their impact on the properties of matter.
IV.Mixtures and Pure Substances
This section distinguishes between mixtures (heterogeneous and homogeneous) and pure substances (elements and compounds). It uses real-world examples like Italian dressing and granite (heterogeneous) to illustrate the differences. The chemical composition of everyday items like smartphones is also examined to showcase the wide application of chemical principles in technology.
1. Defining Mixtures and Pure Substances
This section establishes the fundamental difference between mixtures and pure substances. A mixture is defined as a combination of two or more types of matter present in varying amounts, separable through physical changes such as evaporation. The text highlights heterogeneous mixtures, where composition varies from point to point, providing examples such as Italian dressing (where oil, vinegar, and herbs separate), chocolate chip cookies (with visible chocolate chips and nuts), and granite (with distinguishable quartz, mica, and feldspar). This section establishes the key characteristics of mixtures, emphasizing their variable composition and the ability to separate their components using physical methods. The contrast with pure substances—elements and compounds—is implied but not explicitly defined in this section, leaving further elaboration for later parts of the text. The clear and concise definitions, coupled with illustrative everyday examples, make this fundamental concept of matter readily accessible to the reader.
2. Mixtures in Everyday Life The Example of Smartphones
The section expands upon the concept of mixtures by exploring the chemical composition of a common modern device: the smartphone. It is used as a real-world example to showcase the complex mixtures used in technology. The text details the various chemical substances found in a typical smartphone, categorizing them according to their location within the device. For instance, the case and frame consist of polymers (ABS and polycarbonate thermoplastics) and structural metals (aluminum, magnesium, iron), while the display screen utilizes strengthened silica glass with conductive coatings (like indium tin oxide). The circuit board is described as containing a semiconductor material (silicon), various metals (copper, tin, silver, gold), and less common elements like yttrium, praseodymium, and gadolinium. The battery's composition, involving lithium ions, iron, cobalt, copper, and polymers (polyethylene oxide, polyacrylonitrile), is also examined. This detailed analysis highlights the intricacy of material compositions in even seemingly simple objects, underscoring the prevalence of mixtures in modern technology and manufacturing processes. By using the smartphone as an example, this section effectively demonstrates the relevance of chemical mixtures in everyday technology and their significance in the advancement and development of modern devices.
V.Energy and Chemical Processes Exothermic and Endothermic Reactions
This section explores the relationship between energy and chemical changes. It defines exothermic processes (releasing heat, like combustion) and endothermic processes (absorbing heat, like cold packs). The section also discusses the use of solar energy and its storage in systems like the Solana Generating Station in Arizona, illustrating the importance of chemical reactions in energy production and storage.
1. Energy Sources and Transformations
This section begins by highlighting the sun as the primary source of most of the energy we use. It points out the immense amount of solar energy reaching Earth daily—approximately 10,000 times the world's current energy needs—and emphasizes the challenge of efficiently converting and storing this energy for practical, non-polluting applications. The natural process of photosynthesis in plants and bacteria is presented as an example of solar energy capture. The release of this stored energy through the burning of wood or plant-derived fuels like ethanol, as well as the burning of coal and petroleum (fossilized plant and animal matter), is also discussed. This sets the stage for a discussion of how energy is involved in chemical processes. The section provides context by explaining historical energy measurement units (calories) and the modern standard unit (joules), highlighting the interconvertibility of these units. The introduction of the PhET simulation as a tool for exploring energy forms and changes provides a practical and engaging learning resource for the reader.
2. Exothermic and Endothermic Processes Defining and Illustrating
This section defines and differentiates between exothermic and endothermic processes. An exothermic process is described as a change that releases heat, with the combustion reaction in an oxyacetylene torch provided as a clear example; this reaction also produces light, which is evidenced by the flame. In contrast, an endothermic process is defined as a change that absorbs heat, using the example of a cold pack used for treating muscle strains. The cold pack's operation is explained as a reaction between water and a salt (like ammonium nitrate) that absorbs heat from its surroundings, resulting in a cooling sensation. These clear and relatable examples effectively demonstrate the contrast between exothermic and endothermic reactions. The use of real-world applications, like torches and cold packs, makes these abstract concepts more tangible and easier for readers to comprehend. The section implicitly emphasizes the importance of understanding these concepts within the broader context of energy and chemical reactions.
3. Solar Energy and Thermal Energy Storage The Solana Generating Station
This section provides a concrete example of large-scale solar energy utilization—the Solana Generating Station in Arizona's Sonora Desert. It describes how the station uses parabolic mirrors to focus sunlight onto pipes filled with a heat transfer fluid (HTF). The HTF then generates steam to produce electricity and simultaneously heats a molten salt mixture for thermal energy storage. This storage system allows for continued electricity production for six hours after sunset. The choice of molten salts as the storage medium is justified by their properties such as high heat capacity and thermal conductivity. This section effectively illustrates the practical application of understanding energy transfer and storage in chemical systems on a large scale. By describing the operation of the Solana Generating Station, the text highlights the technological and engineering achievements based on the principles of heat transfer and energy storage. The specific details about the station's design and operation not only reinforce the concepts of exothermic and endothermic processes but also illustrate their potential contribution to the development of sustainable energy solutions.
VI.Calorimetry and Metabolism
This section focuses on calorimetry, a technique used to measure heat changes in chemical reactions. It explains how whole-body calorimeters are used to measure metabolism in humans under various conditions and dietary regimes. The nutritional Calorie (kcal) is defined in relation to heat and energy.
1. Calorimetry Measuring Heat Changes
This section introduces calorimetry as a technique for measuring the heat produced or absorbed during chemical reactions. It describes a specific type of calorimeter—the bomb calorimeter—which uses a robust steel container (the 'bomb') submerged in water. The sample is placed inside the bomb, filled with oxygen under high pressure, and ignited by an electrical spark. The heat released by the reaction is absorbed by the water and the steel bomb, and the resulting temperature increase is measured. This temperature change, along with the known heat capacity of the calorimeter, is used to calculate the energy released (or absorbed) during the reaction. The process of calibrating bomb calorimeters is also explained, involving a known reaction (e.g., burning benzoic acid) to determine the calorimeter's heat capacity. This ensures accurate measurement of the heat involved in subsequent experiments. The description provides a detailed technical explanation of calorimetry, focusing on the bomb calorimeter, a specific type commonly employed for precise heat measurements in chemical reactions. This lays the groundwork for understanding how heat measurements are made and used to understand chemical processes. The section explains the methodology, accuracy concerns, and calibration procedures, highlighting the importance of precise measurement in calorimetry.
2. Calorimetry in Biology Measuring Human Metabolism
The section extends the application of calorimetry to biological systems, focusing on the measurement of human metabolism using whole-body calorimeters. It notes that since the first such calorimeter was built in 1899, numerous iterations have been developed, culminating in modern whole-room calorimeters that allow individuals to perform relatively normal activities. This allows for measurements to better reflect real-world conditions. These calorimeters are used to study metabolism under various conditions—different environmental conditions, dietary regimes, and health states (like diabetes). The nutritional Calorie (kilocalorie, or kcal), a unit used to quantify energy derived from food metabolism, is defined as the amount of energy needed to heat 1 kg of water by 1 °C (equivalent to 1000 calories). The application of calorimetry to human metabolism research highlights a direct link between chemical principles and biological processes. The description of whole-body calorimeters and their use in studying human metabolism demonstrates the interdisciplinary nature of calorimetry, connecting chemical principles to biological research and providing a concrete real-world application of the technique. The use of Calories as a measure of metabolic energy intake underscores the everyday relevance of these scientific measurements.
VII.The Periodic Table and Properties of Elements
This section covers the periodic table, emphasizing its arrangement based on atomic number and the periodic law. It highlights the work of Mendeleev and Meyer in developing the periodic table and discusses the significance of atomic mass and the representation of unstable isotopes. The section also briefly introduces different groups of elements (e.g., alkali metals, alkaline earth metals).
1. Historical Development of the Periodic Table
This section traces the history of the periodic table, highlighting the independent contributions of Dmitri Mendeleev (Russia, 1869) and Lothar Meyer (Germany, 1870). Both scientists recognized a periodic relationship among the properties of known elements, publishing tables arranging elements by increasing atomic mass. However, Mendeleev's work is particularly emphasized for his use of the table to predict the existence of yet-undiscovered elements with properties similar to aluminum and silicon. The subsequent discoveries of gallium (1875) and germanium (1886) provided strong support for Mendeleev's predictions. While a dispute over priority existed between Mendeleev and Meyer, the text notes that Mendeleev's contributions are now more widely recognized. This historical overview provides context for the modern understanding of the periodic table and its predictive power. It highlights the scientific process of observation, prediction, and verification, showcasing how scientific theories evolve and are refined through further research and discoveries. The emphasis on Mendeleev's predictive ability serves as a powerful illustration of the periodic table's utility and significance in the field of chemistry.
2. The Modern Periodic Table and Periodic Law
This section describes the modern periodic table and the periodic law. The modern understanding of the periodic relationship is that the properties of elements are periodic functions of their atomic numbers, rather than atomic masses (as initially proposed). The elements are arranged in increasing order of atomic number, with elements having similar properties grouped in vertical columns (groups) and horizontal rows (periods). Each element's box contains its atomic number, symbol, average atomic mass, and sometimes its name. The table is structured with seven periods and 18 groups (using the traditional US group numbering system). This section clearly outlines the fundamental structure and organization of the modern periodic table, clarifying its use as a powerful tool for organizing and understanding the properties of elements. It emphasizes the shift from atomic mass to atomic number as the basis for periodic relationships, highlighting a key advancement in chemical understanding. The structure and information contained within each element's box are described concisely, allowing readers to quickly grasp the table's fundamental organization and functionality.
3. Atomic Mass and Isotopes in the Periodic Table
The final part of this section focuses on the representation of atomic mass in the periodic table, especially for elements with unstable, radioactive isotopes. The text explains that elements like technetium (element 43), promethium (element 61), and most elements with atomic number 84 (polonium) and higher have their atomic masses shown in square brackets. This is because these elements consist entirely of unstable isotopes, and an average atomic weight cannot be reliably determined due to varying isotopic abundances. The bracketed number represents the atomic mass number (and approximate atomic mass) of the most stable isotope. This explanation clarifies the specific notation used in the periodic table for elements with predominantly unstable isotopes, highlighting the nuances of atomic mass determination and the importance of considering isotopic variation. This detailed explanation of the notation helps avoid misinterpretations and underscores the complexity of atomic structure and properties, particularly for radioactive elements. The section connects the presentation of atomic mass in the periodic table with the concept of isotopes, enhancing the understanding of the periodic table as a tool for representing both stable and unstable elements.
VIII.Ions and Polyatomic Ions
This section explains the concept of ions, both monatomic and polyatomic. The importance of memorizing common polyatomic ions for further study in chemistry is stressed. The section also highlights the work of Lee Cronin, a chemist known for his work in complex chemical systems and their application.
1. Monatomic Ions Formation and Charge
This section introduces the concept of monatomic ions, which are ions formed from a single atom. The text explains that these ions carry an electrical charge due to a gain or loss of electrons. An example is provided illustrating how aluminum (Al) forms a cation with a 3+ charge (Al³⁺), becoming an aluminum ion. The formation of an anion is also illustrated; carbon (C) forms an anion with a 4− charge (C⁴⁻), becoming a carbide ion. This section provides a clear definition of monatomic ions and uses concise examples to show how elements gain or lose electrons to form charged species. The focus is on the basic principles of ion formation—the relationship between electron transfer and the resulting charge—and provides a foundational understanding for subsequent discussions of more complex ionic species. This introductory section effectively prepares the reader for a deeper understanding of ionic bonding and the properties of ionic compounds.
2. Polyatomic Ions Structure and Importance
Building upon the concept of monatomic ions, this section introduces polyatomic ions, defined as electrically charged molecules—a group of bonded atoms with an overall charge. These ions are described as acting as discrete units. The section emphasizes the importance of memorizing the names, formulas, and charges of the most common polyatomic ions due to their frequent use in chemistry. The text specifically mentions oxyanions as a class of polyatomic ions containing one or more oxygen atoms. The concluding statement stresses the importance of memorizing these ions, indicating they will be used repeatedly throughout the study of chemistry. This section highlights a key category of ions with more complex structures than monatomic ions. The emphasis on memorization underscores their practical importance in chemical calculations and nomenclature, underlining the necessity of familiarity with these fundamental chemical building blocks. The mention of oxyanions as a specific subclass of polyatomic ions provides a more detailed glimpse into the various types and complexities of these ionic species.
3. Lee Cronin s Research A Case Study in Complex Chemical Systems
This section briefly introduces the work of chemist Lee Cronin, highlighting his significant contributions to the field. Cronin is described as one of the UK's 10 most inspirational scientists, the youngest chair at the University of Glasgow, and a prolific researcher with numerous publications and invited talks. His research focus on complex chemical systems and their technological applications is mentioned, with additional interests in areas such as nanoscience, solar fuels, synthetic biology, and even artificial life and evolution. This inclusion provides a real-world example of a scientist engaged in cutting-edge research related to complex chemical systems. It demonstrates the ongoing relevance of ionic species and their application in advanced technologies and scientific inquiries. The inclusion of Cronin's profile serves as an inspirational and motivational element, showcasing the exciting and impactful career paths available within the broader field of chemistry.
IX.Food Science Applications Flour Milling and Shortening
This section examines the process of flour milling, detailing the steps from grain to finished flour. It also discusses the composition and properties of shortening, including the shift from hydrogenated vegetable oils due to health concerns related to trans fats. The section notes the rise in demand for artisan flours and their unique characteristics.
1. Flour Milling From Grain to Flour
This section details the process of commercial flour milling, contrasting it with simpler household methods. Household mills produce flour in a single step, while commercial mills use a multi-step process to gradually break down the wheat grain. This gradual process ensures minimal mixing of bran and germ with the endosperm. The initial stage involves cracking the wheat with corrugated steel rollers, creating coarse particles. The grain then passes through progressively finer screens, with air currents removing impurities from the middlings (coarse endosperm fragments). Further refinement involves the use of purifiers (cleaning machines with sieves and air currents) to eliminate dust and additional crushing with smooth steel rollers to reduce middlings into flour. The final flour is bleached, stored in bulk, enriched (with thiamine, niacin, riboflavin, and iron), and then either bagged or prepared for bulk delivery. This detailed description of the commercial flour milling process illustrates the complexity and sophistication involved in producing high-quality flour for baking. The text highlights the importance of precise control at each stage to ensure consistent flour quality, despite variations in the initial grain due to weather conditions. The contrast between household and commercial milling methods underscores the advanced techniques employed in large-scale flour production.
2. Flour Blending and Types of Milling
This section discusses flour blending, a process undertaken at the mill to ensure consistent product quality. The sophisticated analysis and testing (including test baking) allow millers and their chemists to provide bakers with high-grade flour. The text mentions that inconsistencies are usually due to baker error, not the flour itself. The impact of weather on grain quality is also highlighted; damp weather can lead to sprouting and 'damaged starch.' The ability of millers to compensate for these variations through analysis and adjustments is emphasized. The section then introduces artisan milling, a method that produces less refined flours with higher nutritional content and fewer additives, noting the increasing consumer demand for these products. Artisan millers, also known as micro-millers, process diverse grains, including spelt, kamut, buckwheat, and non-gluten grains and pulses, offering bakers greater variety. The importance of ingredient quality and traceability for artisan bakers is also stressed. Finally, rye flour is discussed as the only flour besides wheat that can be used to make yeast-raised breads without blending, highlighting its nutritional value comparable to wheat and even superior in some aspects, such as lysine content. The text describes the milling process for rye, emphasizing its softer endosperm and quicker breakdown into flour compared to wheat.
3. Shortening Composition Properties and Health Concerns
This section focuses on shortening, primarily hydrogenated vegetable oil, highlighting its properties and historical context. Since the early 20th century, it has become almost synonymous with hydrogenated vegetable oil. Its properties are similar to lard: both are semi-solid fats with a higher smoke point than butter or margarine, making them less prone to splattering during frying. The higher fat content (close to 100%) compared to butter and margarine (around 80%) is noted. The section discusses 'high-ratio shortenings,' which contain a higher percentage of monoglycerides, blending better with hydrophilic ingredients. However, the section also addresses health concerns related to the traditional use of partially hydrogenated vegetable oils containing trans fats. The reformulation efforts of Crisco and other brands to remove or significantly reduce trans fats are mentioned, highlighting the shift towards healthier alternatives. Non-hydrogenated vegetable shortening made from palm oil is presented as a viable trans-fat-free option. The discussion showcases the evolution of shortening based on changing understanding of its chemical composition and health impacts.
X.Brønsted Lowry Acids and Bases
This section provides an overview of the Brønsted-Lowry definition of acids and bases, contrasting it with the Arrhenius definition. It emphasizes the concept of proton transfer as the defining characteristic of acid-base reactions.
1. Historical Overview of Acids and Bases
The section begins by providing a historical context for the understanding of acids and bases. Robert Boyle's characterization of acids in 1680 is mentioned, noting their ability to dissolve substances, change the color of certain dyes (like litmus), and lose these properties when reacting with alkalis (bases). Eighteenth-century understanding is summarized, highlighting the sour taste of acids, their reaction with limestone to produce CO₂, and their interaction with alkalis to form neutral substances. Humphry Davy's 1815 contribution is noted, demonstrating hydrogen as an essential component of acids. Joseph Louis Gay-Lussac's contemporary work on acid-base neutralization is also mentioned, emphasizing the mutual definition of acids and bases. Finally, Carl Axel Arrhenius's 1884 definition of acids and bases in terms of hydrogen cations (hydronium ions) and hydroxide anions in aqueous solutions is discussed. This historical overview sets the stage for the introduction of a more comprehensive model, the Brønsted-Lowry definition, illustrating how scientific understanding evolves and expands over time. The mention of key historical figures and their contributions enhances the reader's appreciation for the development of acid-base theory.
2. The Brønsted Lowry Definition of Acids and Bases
This section introduces the Brønsted-Lowry definition of acids and bases, proposed in 1923 by Johannes Brønsted and Thomas Lowry. This definition centers on the proton (H⁺), defined as what remains of a hydrogen atom after losing an electron. A Brønsted-Lowry acid is defined as a compound that donates a proton to another compound, while a Brønsted-Lowry base is a compound that accepts a proton. An acid-base reaction, therefore, is characterized as a proton transfer from a proton donor (acid) to a proton acceptor (base). This definition is presented as a more general and inclusive model compared to the earlier Arrhenius definition. While acknowledging that the Arrhenius definition is not inherently incorrect, it's described as limited. This comparison helps to highlight the advantages of the Brønsted-Lowry model in explaining a wider range of acid-base reactions. The clear definition and explanation of the proton transfer mechanism at the heart of Brønsted-Lowry acid-base reactions makes the concept easy to understand, while also emphasizing its broader applicability compared to the previous Arrhenius definition. The text concludes by hinting at a more general model, the Lewis definition, which will be introduced later in the text.